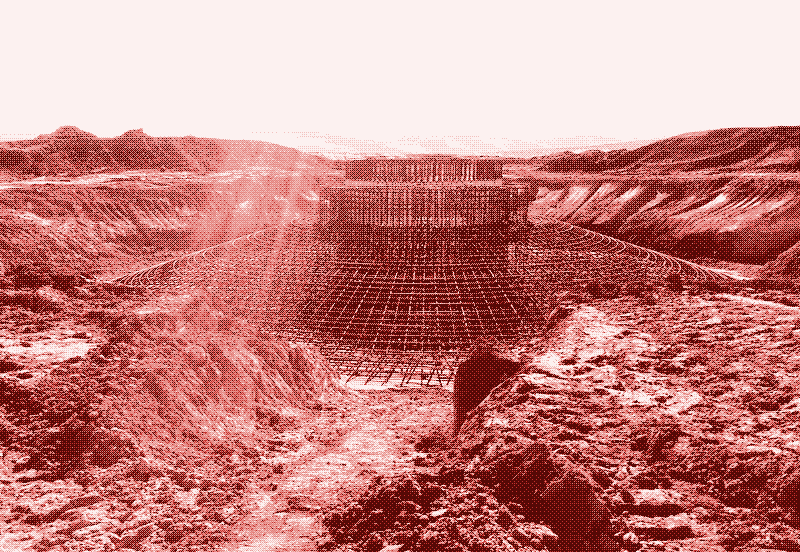
Trapped in the Iron Age
In 1836, Danish antiquarian and curator Christian Jürgensen Thomsen distinguished three prehistorical eras based on the dominant materials used for weapons and cutting implements: the Stone Age, the Bronze Age, and the Iron Age.1 Thomsen’s classification refers to the past, but according to his criteria, we have never evolved beyond the Iron Age. Even in the 21st century, iron remains the dominant material, not just for weapons and cutting implements but for about every modern technology.
We now use most iron in the form of steel. However, according to Thomsen’s criteria, we cannot speak of a “Steel Age.” First, steel is merely an alloy of iron (>98%) and carbon (<2%). Second, humans have been producing steel since the beginning of the Iron Age. That is a little-known fact in the Western world, where steel production only took off in the nineteenth century with fossil fuels. However, Asian and African metallurgists developed high-quality steels much earlier, and this knowledge eventually allowed Europeans to do the same – on a much larger scale.2
By 2021, the global iron and steel output reached 1,950 million tonnes (Mt). That is 22 times larger than the combined aluminum and copper output (88 Mt). The global iron and steel output corresponds to five times the global plastics output (391 Mt) and dwarfs the worldwide production of silicon (8.5 Mt) and lithium (0.1 Mt).34 Steel is the fundamental material of industrial societies. Without plastics, lithium, or silicon, we would still be in an industrial society. Without iron and steel, we would be thrown back 3,000 years into the Bronze Age.
Where is all that steel?
The massive presence of steel in industrial society is not so obvious.5 At home, we find several steel appliances such as the refrigerator, washing machine, water boiler, bathtub, and cooking, heating, and cooling appliances. However, only 2-3% of total steel production ends up in domestic appliances.678 Outdoors, there’s a lot of steel in the form of vehicles. These are especially passenger cars that use around 10% of all steel globally (20% in rich countries). Busses, trucks, trains, and ships add another 4-5%. Altogether that is still less than 20% of the global steel output.
Most steel is embedded in other materials, located underground, or far away from residential areas.
Most steel is embedded in other materials, located underground, or far away from residential areas. More than half of global steel production goes into construction, which includes buildings (residential, commercial, industrial) and infrastructures (bridges, tunnels, harbors, canals, runways, oil rigs, refineries, pipelines, power plants, transmission lines, railways, subways, and so on). Much of that steel is embedded in concrete. Reinforced concrete is the world’s primary building material, and concrete is the only material that can match the output of steel (1,819 Mt in 2021).
Roughly 15% of global steel production serves to make machinery, including machine tools, industrial equipment, electrical hardware, and construction, mining, and farming machines. Even products made of other materials – such as other metals, plastics, and wood – are shaped by steel tools.5 The final 15% of steel production ends up in a variety of objects, from screws over food packaging to furniture and shipping containers.678
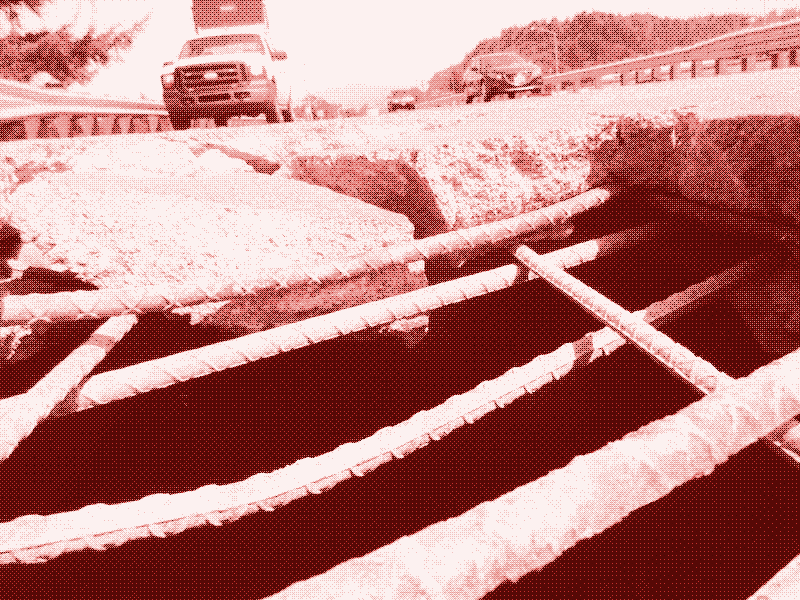
The environmental footprint of the steel industry
Steel is often presented as one of the most sustainable materials. Unlike plastics, steel can be recycled without any loss in quality. The steel industry has made great advances in energy efficiency, more so than many other industries. Making one ton of crude steel now requires roughly 20 gigajoules (GJ) of primary energy on average – three times less than in 1950.9 This compares very favorably to other materials such as aluminum (175 GJ/t), plastics (80-120 GJ/t), or copper (45 GJ/t).7 Unlike plastics, steel is a biodegradable material.10 Finally, iron ore is not in short supply. It makes up 5 percent of the Earth’s crust and is fourth in abundance among the elements.11 For comparison, copper only makes up 0.01%.5
However, despite all these advantages, the global iron and steel industry consumes more energy and produces more carbon emissions than any other industry. The total primary energy use of crude steel production was 39 exajoules (EJ) in 2021, which corresponds to 7% of all energy used worldwide in that year (595 EJ). The greenhouse gas emissions are even higher because around 75% of energy use comes from coal – the fuel with the highest carbon emissions. In 2021, the iron and steel industry produced 3.3 Gt of carbon emissions, roughly 9% of global emissions (36.3 Gt).12 The concrete industry follows closely with 8%.
The iron and steel industry consumes more energy and produces more carbon emissions than any other industry.
The estimates above come from the World Steel Association and the International Energy Agency. These data are available for all metals and have been documented over a long period, allowing for historical comparisons. However, they only refer to the smelting of the metal. They do not include the energy use and carbon emissions for mining and transporting iron ore, coal, limestone, scrap, and steel products. Nor do they include the energy and emissions for coke production and ore preparation – all essential to the steel production process.7
Scientific studies that have set wider boundaries for the iron and steel industry conclude that the energy cost of steel production increases by 50% to 100%.13 One report concludes that the methane emissions from metallurgical coal mining alone could increase emissions by 27%. Another study estimates that seaborne transport of iron ore and steel adds 10-15% extra emissions.1415 Iron and steel production also create other environmental problems, such as high water use, solid waste production, and significant air and water pollution.
The carbon footprint of the iron and steel industry is incompatible with current ambitions to eliminate net carbon emissions by 2050, even less so because steel production is very likely to expand further. Steel production grew tenfold since 1950 and doubled between 2000 and 2020, growing faster than many researchers had predicted.16 Furthermore, efficiency gains have decreased, and there is a scientific consensus that current technologies have reached their thermodynamic limits.7917 During the last two decades, the average energy use for the production of 1 ton of steel has remained around 20 GJ/t.918
How to make steel without fossil fuels?
There are two ways to make steel, and one is much more sustainable than the other.19 On the one hand, there is the blast furnace or basic oxygen furnace, in which steel is made from iron ore and coal. This technology is – in its essential form – 2000 years old. On the other hand, there is the electric arc furnace, in which steel is made from steel scrap and electricity. The electric arc furnace, which is a relatively new technology, consumes much less energy than the blast furnace, makes use of a recycled resource (no need to mine iron ore), and works without the direct use of coal or other fossil fuels (the electricity can be supplied by solar, wind, or atomic power).
The most energy-efficient electric arc furnaces now consume less than 300 kilowatt-hours of electricity per ton of steel produced.920 Hypothetically, if we had produced all steel in 2021 (1,950 Mt) in such furnaces, the total power consumption of the global iron and steel industry would have been only 585 terawatt-hours (Twh). That corresponds to just one-third of all electricity generated by wind turbines worldwide in the same year (1,848 Twh). Unfortunately, more than 70% of global steel output was made in blast furnaces fed by coal and iron ore.920 A blast furnace consumes twenty times more energy and cannot be operated by electricity because coal is both the fuel source and the chemical reductant. The combustion of coal produces carbon monoxide that reduces the iron from its ore.7
Not enough scrap available
The solution seems obvious: let’s produce all that steel in electric arc furnaces. However, this is impossible. There’s not enough scrap available: the continuous growth of the global steel output makes a circular flow of resources impossible.21 It takes decades before most steel becomes available for recycling. For example, there is 543 Mt of steel stocked in ships.22 The scrap available for recycling in 2021 corresponds to the production level of 1965 when global steel production was less than one-quarter of what it is today (450 Mt).9101523 Consequently, the other three quarters need to be produced in blast furnaces using coal and freshly mined iron ore.
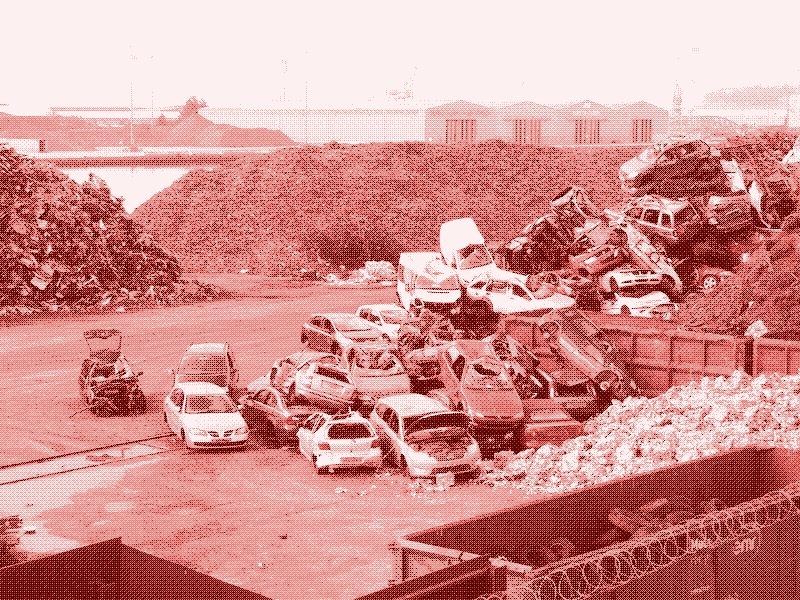
Nowadays, China produces roughly half of the steel in the world and does that almost exclusively (+90%) in blast furnaces using coal and iron ore. Many other steelmaking nations have a higher share of electric arc furnaces. However, it makes little sense to point the finger at China. First, the US and Europe have outsourced many of their industries to China since the 2000s, a trend that corresponds neatly with the growing steel output in that country. Furthermore, twenty to forty years ago, China hardly used any steel. Consequently, there is almost no scrap available. China has no other choice than to use blast furnaces.24
Ever higher grades of steel
A second obstacle is the continuous development of higher grades of steel. There are now over 2,500 different types of steel with a variety of properties, such as increased strength, tolerance to high temperatures, or corrosion resistance.792325 Although these higher quality steels can be produced in electric arc furnaces, they are not made from scrap, and they have much higher energy use.
Steel available for recycling forms a mix of steel grades. That mix is suitable for making plain carbon steel but not highly alloyed steels, which require scrap with similar qualities. However, that scrap is not available. For example, stainless steel, the most produced special steel grade, has a recycling rate of only 15%. Almost 60 Mt of stainless steel was produced in 2021, compared to only 4 Mt in 1980.26 The traditional use of stainless steel was in cutlery, surgical tools, and medical and food processing equipment. However, it is now also used in the construction of tunnels and outdoor furniture, wastewater treatment, seawater desalination, nuclear engineering, and the production of biofuels.7
The low recycling rate and the need for the extraction of additional elements such as chrome and nickel make higher grades of steel more energy-intensive to produce. For example, stainless steel production requires almost 80 GJ per ton, four times more than the production of plain carbon steel.723 The continuous development of higher-grade steels is stimulated by environmental legislation (such as the use of lighter steel in cars) and by competition from other materials, mainly aluminum and plastic composites.792325 Ironically, the competition with these materials, which consume even more energy, makes steel less and less sustainable.
Steel and renewable energy
The steel industry is heavily dependent on the energy supply, but the energy supply is also heavily dependent on the steel industry. Almost 10% of the global steel output goes into building and maintaining energy supply infrastructure. That amount corresponds to the entire steel output in 1950. A great share of that steel goes to gas and oil infrastructure.27. Oil and gas mining, production, and transportation require steel for offshore drilling platforms, pipelines, refineries, tankers, and storage tanks. Coal mining depends on steel for cutters, loaders, conveyors, excavators, and trucks.7
Unfortunately, the planned switch to low-carbon energy sources and the electrification of heating and transport technologies will not decrease our dependency on the steel industry – on the contrary. A low-carbon power grid requires much more steel (and other materials) than an infrastructure based on fossil fuels. Wind and solar power are very diffuse power sources compared to fossil fuels. Therefore, it takes much more materials (and land) to produce the same energy. In jargon, wind and solar have low “power density” or high “material intensity.”2829303132
A low-carbon power grid requires much more steel than an infrastructure based on fossil fuels.
The “steel intensity” of thermal gas and coal power plants is between 50 and 60 tonnes of steel per megawatt of installed power.33 Hydroelectric power plants have a lower steel intensity, with 20-30 tonnes of steel per MW.733 Atomic power’s steel intensity is also lower at between 20 and 40 tonnes of steel per installed MW.3334 On the other hand, solar PV requires between 40 and 170 tonnes of steel per installed MW.3335 Although there is little or no steel in the solar panels themselves, it’s the material of choice for the structures that support them.
Steel and wind power
The most steel-intensive power source – by far – is the modern wind turbine. The steel intensity of a wind turbine depends on its size. A single, large wind turbine requires significantly more steel per megawatt of installed power than two smaller wind turbines.36 For example, a 3.6 MW wind turbine with a 100-meter tall tower requires 335 tons of steel (83 tons/MW), while a 5 MW wind turbine with a 150-meter tall tower needs 875 tons of steel (175 tons/MW).37 The trend is towards taller wind turbines and a higher steel intensity.
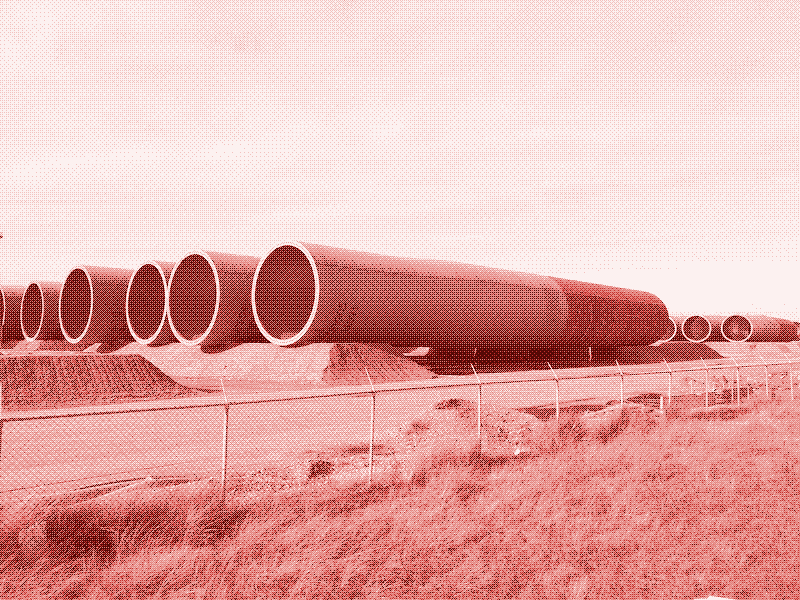
Steel consumption further increases for offshore wind turbines. Onshore wind power plants rely on reinforced concrete for their foundations, but offshore wind turbines need massive steel structures such as monopiles and jackets.38 The steel intensity for offshore wind turbines is calculated to be around 450 tonnes per MW for a 5 MW turbine – eight times higher than the steel intensity of a thermal power plant.36. As these wind turbines get taller and move into deeper waters, their steel use further increases.
The most popular offshore wind turbine nowadays has a capacity of 7 MW, while the largest ones have a capacity of 14 MW.36 If we make a conservative estimate based on the data above (the steel intensity doubles for every doubling of the power capacity), a 14 MW offshore wind turbine would require 1,300 tons of steel per MW or 18,200 tonnes in total. Such a wind turbine thus consumes 24 times more steel than a coal or gas power plant of the same power capacity.
Shorter life expectancy
The difference between renewable power sources and fossil fuels becomes even larger if the steel intensity is calculated per unit of energy rather than power (MWh instead of MW). In contrast to coal and gas power plants, the output of wind and solar power plants depends on the weather, and they do not always produce their maximum power capacity. Therefore, replacing 1 MW of fossil electricity generation capacity requires the installation of (on average) 4 MW of solar power or 2 MW of wind power.39 A 14 MW offshore wind turbine thus has a steel intensity that is almost 50 times higher than a fossil fuel power plant for every kilowatt-hour of electricity produced.40
A 14 MW offshore wind turbine has a steel intensity that is almost 50 times higher than a fossil fuel power plant for every kilowatt-hour of electricity produced.
Solar and wind power plants also have shorter lifetimes (20-30 years) compared to thermal power plants (30-60 years).31 While this does not affect the steel intensity per MW of power installed, it again increases the steel intensity per unit of energy produced over time. That does not always lead to a doubling of steel use because foundations for offshore wind turbines and structures for solar panels may have longer lifetimes than the power sources they support and could thus be reused.41
Power transmission infrastructure
The data above only include the steel used in the power plants themselves. For fossil fuel power plants, they do not include the steel used in the pipelines, oil rigs, coal excavators, and the like. However, the same goes for the low-carbon power sources. Because they need much more resources than thermal power plants (steel but also other metals and materials), they depend on a global mining and transport infrastructure that is just as steel-intensive as the supply chain for fossil fuels.
Furthermore, because they are more diffuse power sources with intermittent and unpredictable power production, often located far away from energy consumption centers, renewable power plants drive the expansion of transmission infrastructure. That infrastructure is also based on steel – from switchyard equipment over towers to conduction cables.282930313242
Finally, low-carbon power sources also have a high need for special grades of steel, which are more energy-intensive to produce. Steel for off-shore wind turbines should resist corrosion, and stainless steel is increasingly used for solar panel support structures.43 Electrical lamination steel (iron-silicon) is indispensable for transformers in the power network.7 Nuclear power plants may have a relatively low steel intensity but are completely built up of energy-intensive specialty steels. For example, cladding the fuel elements containing fissionable uranium requires zirconium steel, while all structural elements contain austenitic stainless steel.744
Low carbon grid cannot be made from recycled steel
The high steel intensity of low carbon power sources confronts us with a so-called “catch-22”, a situation in which there seems to be no escape from a problem no matter what we do. We need much more steel if we replace thermal power plants with renewable ones. Because there is not enough steel scrap available, we can only produce that extra steel from iron ore in blast furnaces burning fossil fuels. To address climate change, we need to build low-carbon sources quickly and in great numbers. However, to achieve circular material flows and build low-carbon power sources from scrap and renewable electricity, we would have to do the opposite: slow down the development of a low-carbon power grid.
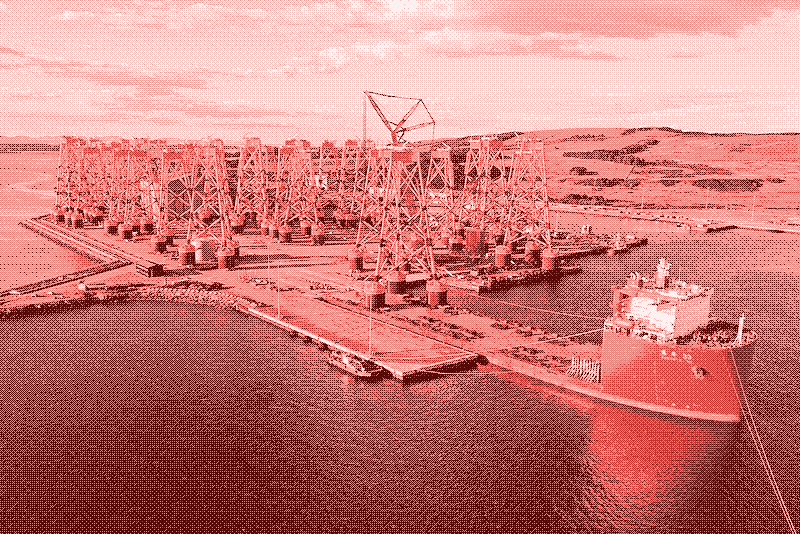
A well-cited study from 2013 concluded that if wind and solar power would supply 25,000 Twh of electricity – which corresponds to total global electricity demand in 2021 – we need about 3,200 Mt of steel to build the power plants alone.3345 Global electricity demand is projected to grow to between 52,000 and 71,000 terawatt-hours in 2050, which would increase the extra steel demand to between 6,400 and 8,960 Mt.46 Spread out over the lifetime of solar panels and wind turbines (25 years), we would have to produce 256 to 358 Mt extra steel per year to make wind turbines and solar panel structures – comparable to the steel demand for passenger cars (195 Mt) and other transportation modes (98 Mt) combined.
That is still a very optimistic estimation. Electricity demand only makes up around 20% of total energy demand. If the total energy demand (177,000 Twh in 2021) would be supplied by wind and solar, we would need 22,400 Mt of steel. That’s an extra 896 Mt steel per year – as much as the global production in the early 2000s. You could argue that electricity can be used more efficiently than fossil fuels, for example, in cars and heating systems. However, at the same time, total energy demand is expected to rise further, countering the gains made by increased energy efficiency.
The high-tech solutions
The steel industry counts on technological solutions to make steel production carbon neutral. One option is to replace coal by gas, an approach that is already common in the Middle East and North America. Gas-based steelmaking results in somewhat lower carbon emissions, but they are still much higher than in the case of the electric arc furnace. Therefore, most attention goes to hydrogen, which can replace purified coal (coke) as a reducing agent in a direct reduction shaft furnace.47 However, hydrogen-based steelmaking does not offer an escape from the catch-22 because it further increases the need for a steel-intensive infrastructure.
The production of hydrogen is energy-intensive. It takes 50-55 kilowatt-hour to make 1 kg of hydrogen and 60 kg of hydrogen to make 1 ton of steel.47 The production of 1 ton of steel from hydrogen thus consumes 3,000 kWh of electricity, which is ten times higher than the electricity use of an electric arc furnace making steel from scrap. Consequently, hydrogen-based steelmaking requires roughly ten times more wind turbines and solar panels than scrap-based steel production – and thus ten times more steel. On top of this comes the steel for building the pipelines and storage tanks that are part of the hydrogen infrastructure.
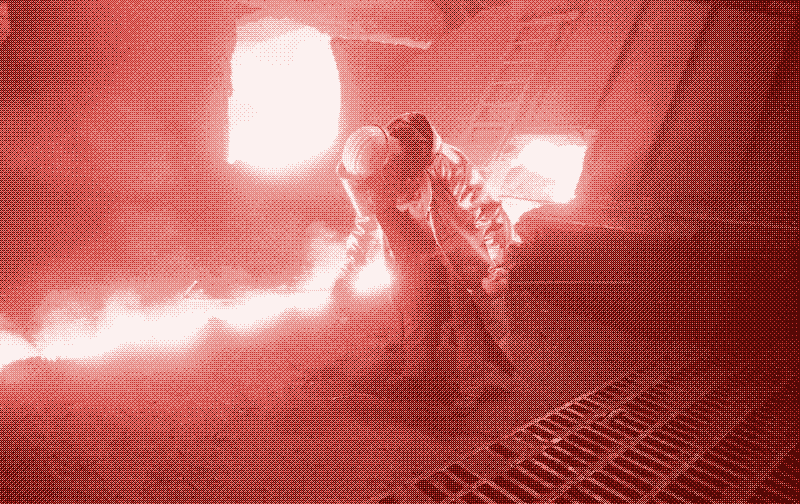
Carbon capture and storage, in which the carbon emissions of steelmaking plants are captured and then stored underground, faces the same problems. It requires a steel infrastructure and extra energy, thus indirectly raising the use of fossil fuels. Reverting to older, preindustrial steelmaking processes is not the answer either. Today’s blast furnace is essentially still the blast furnace from earlier centuries, only much more energy efficient.7
The low-tech solutions
The picture painted above seems to offer little hope for carbon-neutral steelmaking and power production. However, there is a low-tech solution that could achieve it. We could adjust steel production to the available scrap supply both in quantity and quality. That would allow us to produce all steel from scrap in electric arc furnaces, dramatically reducing energy consumption and eliminating almost all carbon emissions. Of course, the intent should not be to replace steel with plastic composites and aluminum because they are even more energy-intensive to produce. The only solution is to reduce material use overall.
We could adjust steel production to the available scrap supply both in quantity and quality.
Reducing the steel output and using more common steel grades would not bring us back to the Bronze Age. As noted, global end-of-life ferrous scrap availability was approximately 450 Mt in 2021, which would allow us to produce roughly one-quarter of the current steel output. Furthermore, the scrap supply will continue to rise for the next 40 years, enabling us to produce more and more low-emission steel each year. By 2050, scrap availability is expected to rise to about 900 Mt, almost half of today’s global steel production.48 All that extra steel could be invested in expanding the low-carbon power grid without raising emissions first.
There is a lot of room to reduce the steel intensity of modern society. All our basic needs – and more – could be supplied with much less steel involved. For example, we could make cars lighter by making them smaller. That would bring energy savings without the need for energy-intensive high-grade steel. We could replace cars with bicycles and public transportation so that more people share less steel. Such changes would also reduce the need for steel in the road network, the energy infrastructure, and the manufacturing industry. We would need fewer machine tools, shipping containers, and reinforced concrete buildings. Whenever steel intensity is reduced, the advantages cascade throughout the whole system. Preventing corrosion and producing steel more locally from local resources would also reduce energy use and emissions.1014
The continuous growth of the steel output – the increasing steel intensity of human society – makes sustainable steel production impossible. No technology can change that because it’s not a technological problem. Like forestry can only be sustainable if the wood demand does not exceed the wood supply, steel is sustainable or not depending on the balance between (scrap) supply and (steel) demand. We may not be able to escape the Iron Age, but we have an option to escape the catch-22 that inextricably links steel production with fossil fuels.49
Reactions
To make a comment, please send an e-mail to solar (at) lowtechmagazine (dot) com. Your e-mail address is not used for other purposes, and will be deleted after the comment is published. If you don’t want your real name to be published, sign the e-mail with the name you want to appear.
Reactions
Unit 87
This is the most coherent, informative, and rational approach to the “climate change” discussion I have encountered to date.
I was reminded of an enlightening discussion I had some 40 years ago with a friend who worked with the National Crushed Stone Association magazine. I had innocently asked him if his job was interesting, thinking that “crushed stone” was not a really hot topic. His dry response was, “Crushed stone is possibly the oldest and largest industry in the world. Concrete is made of crushed stone. How dull can it be?” I was slack-jawed, as my understanding of the world began to grow by orders of magnitude.
Since then, until now, I have never been offered a perspective on climate change that addressed the problem with the fundamental requirements of modern human life on Earth in their proper order of priority, let alone with an accurate awareness of magnitude.
Thank you.
Caroline
Hello Kris,
Another superb article, honestly confronting reality, this time where steel use is concerned. Who wants degrowth though? People seem to be more willing to face the certain terrible unfolding consequences of maintaining expansion.
One glaring omission in scope involves arms, military tanks, planes, bases, and other war-related equipment, for which demand is rising sharply, along with tech complexity. They require massive amounts of steel, energy and so on. Estimates of emissions for the military sector ran at about 5% of total a few years ago, when the Kyoto Agreement and national security excuses allowed information access to be curtailed, resulting presumably in estimates very much on the low side. To me, militarism encapsulates everything humanity needs specifically not to be doing at this historic juncture: wasting and spoiling resources purposed for destruction, genocide, ecocide etc.
Thank you for your original thoughtful work, which is a shining light in the gloom.
Best,
Caroline Ireland
Brady Heyen
Another fantastic writeup, Kris. Once again, a hard look at the data reveals that many of the “hard problems” of our global civilization have a very simple solution: reduce consumption. Thankful for your energy-efficient writing style that allows a simpleton like myself to get a basic understanding of such a complex topic like steel.
Brady Heyen
Jonathan Hartnell-Beavis
Thank you Kris for a thoroughly researched and revealing article. It seems that most solutions to our problems emerge from a reduction in use and demand rather than the inexorable expansionism we seem trapped in. Will we ever learn……..? Presumably only when the cost of carbon is sufficiently high to deter additional consumption -eg there is a negative marginal utility attached to increased consumption through a taxation mechanism. Keep up the great work!
Jonathan Hartnell-Beavis
Thijs Bollen
Thank you Kris for another eye-opener. But, evidence based or not, and with all respect and admiration for your work, your conclusions are getting… boring, namely that we must reduce our consumption. However true that may be, it is, more than 50 years after the Club of Rome, simply not happening, on the contrary: we are growing and we want growth. We are creators by essence. Of course we CAN choose to create more immaterial things such as social happenings, listening sessions, theather plays and so on, which will not only provide an escape to the catch 22, but will very likely also give us more happiness and more peace than the purchasing of the majority of all Amazon-crap. And we CAN choose to create newly designed products with scrap or other waste products, but all this is definitly not what motivates the majority of people. The majority of the world population wants to raise their social position by obtaining material symbols, and who can say they won’t have the right to get up to standards which we in the rich countries are enjoying since decades? For me, THIS is the real catch 22: we all want what only the few have. And we want that because we see this difference as an inequity, that is as something unjust and unfair. And we see inequity because we cannot accept inequality. But even as, for example for religious reasons, not only the rich, but also the poor will start seeing inequality AND its consequences not only as something natural but also as something that is ethically neutral, then we still are creators that want to give meaning to their lives, that want to develop themselves, that want… to grow. And this ETHICALLY FOUNDED striving, in whatever form, not only costs (natural) resources, but also causes social dynamics and thus (natural) resources again.
So where does this leave us? Waiting until we are getting more direct wars over resources and severe economic crashes and so de-grow the hard way? Or do we have to negate ethics, that is to negate the urges of many many people and massively have to turn into Kris’ disciples and preach his material minimalism? Or ask our elites, that is, in this context, ourselves, to de-grow by redistributing our wealth? But even if this kind of huge leap in charity will be possible this will inbalance our public AND personal economies while there is no safety net in sight.
I don’t see the technology-independence related to this issue as Kris describes it, because how can we draw future related conclusions when we cannot know what we don’t know yet? Yes, decarbonized energy production and infrastructure is NOW a catch 22, but, for example: what will the steel intensity be from yet to be realized nuclear fusion reactors? What would a tripling of the efficiency of solar cells yield in this context? Which inventions can we expect in iron/steel production process chemistry?
We are all screen-addicts. And so for me it will be difficult, but also very welcome when energy, internet and stuff is not always available. When I know when I can expect the availability and inavailability, I can adjust my rhythm of life activities according to this pattern. And when we can all agree on such a pattern that does not make a distinction between social-economic classes, we can all feel ethically justified too. But now I’m getting very idealistic… Much more practical and effective are tax policies. But which politicians will have the courage for this?
But we surely will reach nowhere when we will allow pessimism in our minds. Elon musk has turned into a very scary and to be feared power mastodont, but his ‘idiot’ dreams ARE the spirit that we need.
I am curious to hear reactions!
Thijs Bollen.
Alan Borer
A Truly Outstanding Article About the Continuing Iron Age—Your Wrap-up is Nonsense.
Hello Kris:
Too many people on the planet.
All the above “too many people” want more goods and services NOT less.
More public transit with better interconnectedness will help but most people want the convenience of a POV ( cars and motorbikes ) and soon there will be
personal helicopters.
If the current emissions of humans get cut way down (which will not happen) the massive amounts of CH4 and CO2 coming from the melting Arctic permafrost and emanating from under the Antarctic cannot be stopped.
FINITO
Alan Borer
Denis
Hi Kris,
Your article offers a good analysis of the steel problem. I’d like to bring to your attention that it builds on shaky foundations.
As you may or may not be aware, carbon accounting counts land use emissions as carbon stock changes. This sweeps a class of net emissions that can be curbed with simple land stewardship changes out of view as the attached paper puts forward.
Those dwarf energy emissions, so the energy signature of making (and, I’d argue, using) steel actually matters little. If memory serves me well from back when I dove into your work, you are well versed in number crunching and technical matters, so I’m sure you’ll be able to make sense of and evaluate what the paper lays out.
That still leaves a steel issue, of course. But it’s one of not stealing lands from indigenous peoples (which is what carbon offsets enable in practice) to open new mines (and grow tree plantations); and suppressing the world’s biggest consumer of steel and other metals (which is the military industrial complex, see https://londonminingnetwork.org/wp-content/uploads/2020/04/Martial-Mining.pdf).
Best, Denis
M. E. Selous
Dear Kris,
Thanks for the thought-provoking post. Unfortunately, industrial materials are an oft-overlooked subject in the energy transition discourse. I agree that we can gain much from reducing our overall demand for steel. After reading some of your estimates, however, I felt that I had to comment as in my opinion they grossly misrepresent the scale of the challenge we are facing.
You cite a 2013 study from Vidal, Olivier, Bruno Goffé, and Nicholas Arndt, which concluded that increasing wind and solar energy production by 24,600 TWh would require about 3,200 Mt of steel. This figure immediately stood out to me as surprisingly large. So I did a quick back-of-envelope calculation.
—> According to Statista, building 1 MW of solar PV required 56 tons of steel in 2017. The Statista figures for building 1 MW of onshore and offshore wind date to 2018 and are 142 tons and 117 tons respectively. These quantities are surely an overestimate for 2024, what with the continuing improvements in solar PV and wind turbine performance. But let’s be generous here and estimate it takes 60 tons of steel to build 1 MW of solar PV and 150 tons of steel to build 1 MW of wind capacity.
—> In its 2023 world energy outlook, the IEA provides estimates of future renewables capacity. Its net-zero emissions by 2050 scenario (NZE) calls for 17,600 GW of solar and 6,700 GW of wind capacity addition. That represents an addition of roughly 50,000 TWh of yearly renewable energy generation, or double the goal chosen in the 2013 study by O. Vidal et al. How much steel would this require?
—> 60 * 17,600 * 10^3 + 150 * 6,700 * 10^3 = 2,061 Mt of steel
—> For 200% the amount of energy production, we get a requirement for steel that is 36% lower than O. Vidal et al.
Of course, this is just a quick calculation that doesn’t take into account the steel that might be consumed by an expansion of the electricity grid or of battery storage, for instance, both of which would be required by an expansion in renewable capacity. But do these additional factors make steel requirements increase by a factor of three?
The Energy Transitions Commission (ETC) released a 130-page report in July 2023 looking at just this question. The report is titled the “Material and Resource Requirements for the Energy Transition”, and it adopts a holistic approach that estimates the material needs for the transition as a sum total of all required additions to the energy system. (Wind and solar power generation capacity, but also nuclear plants, electricity grid upgrades, storage solutions, hydrogen production, etc.) Its net-zero scenario includes 87,000 TWh of wind and solar production, 57% more than the IEA’s NZE scenario. This report by the ETC estimates that cumulative steel demand for the energy system transition will be between 3,700 Mt and 4,900 Mt (depending on how optimistic one’s outlook is on efficiency gains and technological innovation.)
This is a far cry from the figure of 22,400 Mt that you arrive at in your post. Part of the reason your estimate is so inflated is because it is based on tenuous data. The study you cite by O. Vidal et al., which provides the basis for your steel demand calculations, is over 10 years old. There has been much evolution in wind turbine and solar PV technology since then. And at less than three pages long, I didn’t find anything in this study approaching a methodology section that might explain how the authors arrived at their findings.
Your target for a low-carbon energy system is also unnecessarily ambitious. There’s no reason to require a 100% solar and wind mix. The IEA’s NZE scenario envisions that in 2050, 71% of energy production will be renewables, a little over half of which will be made up of solar and wind, with 12% covered by nuclear and the remaining 17% by fossil fuels (with and without CCUS). Dump the 17% fossil fuels and replace them with wind and solar, and you have a close to irreproachable energy mix, with assumptions for renewable capacity installment that are still below those of the ETC.
Perhaps you might contest the authority of the IEA or the ETC on the subject. Fair enough. No study or report is perfect, and predicting future steel demand is an uncertain endeavor. So I went looking in O. Vidal’s publishing history. There is in fact a much more detailed and recent paper, dating from September 2022, which analyses the steel requirements for the energy transition and on which O. Vidal is a co-author. The paper is titled: “Dynamic modeling of global fossil fuel infrastructure and materials needs: Overcoming a lack of available data.“ In it, the authors estimate that achieving the IEA’s NZE scenario would only require… 1,000 Mt of cumulative steel demand by 2050!
How do they arrive at such a low figure? The authors assume that a significant amount of the steel embodied in the current fossil fuel energy system will be recycled to produce new green energy infrastructure. In other words, they adopt the exact circular economy approach you argue for in your post, but that you selectively apply only to a “low-tech” scenario in order to suit your argument. (Are EAFs really low-tech?)
What I’m trying to point out is that you are intentionally blowing the figures way out of proportion in order to rule out any new steel production. You make some excellent points about the benefits that would come from reducing steel intensity in our modern society. I can only agree. Steel production accounts for anywhere between 5% and 11% of global emissions, and many energy-intensive products are steel-intensive. Greater sobriety in steel consumption will make the transition towards a sustainable energy system much easier. But if we are to build renewables at scale, we need steel now. And for a short while, that steel will be produced from fossil fuels. There is no avoiding this. But by building large amounts of renewables, it is then possible to attain low-carbon steel production within a low-carbon society.
And yes, low-carbon steel production is possible. You willfully misrepresent future pathways for steel production as a binary choice between scrap-based production or production using only hydrogen direct reduction. This makes little sense as the two technologies are not only compatible, but mixing scrap with direct reduced iron is in fact the norm in EAFs across the industry today (rather than production based on 100% scrap.) But more importantly, as you mention yourself in your post, a blast furnace consumes twenty times more energy than an EAF furnace. So even if hydrogen-based production consumes ten times more energy than scrap-based production, replacing blast furnaces with hydrogen-based production would still amount to a halving of energy use! (This is in line with the IEA’s projections, which expect an overall reduction of a quarter in the energy consumption of the steel industry by 2050).Your theory of an inescapable catch-22 that “inextricably links steel production with fossil fuels” has no basis in fact.
In the end your argument is a narrow one. You are uncomfortable with the concept of energy transition. Specifically, the fact that building a low-carbon energy system will require a temporary increase in carbon emissions now, in order to secure much lower emissions later. To defend this position, you hugely overstate the steel requirements that will be needed by the energy transition. You then make the unsupported claim that low-carbon steel production is entirely impossible without fossil fuels. All this to set up a false dilemma between virgin steel production and a sustainable future. As I hope I have shown, the horizon of sustainable possibilities offered to us is much wider.
Yours sincerely,
M. E. Selous
Kris De Decker
@ M.E. Selous
First of all, thanks for your feedback. I appreciate that readers dive into the references and hold the data up to the light. You raise a lot of points so I will address them one by one below.
You cite data from Statista for the steel intensity of wind turbines and solar panels and call them “surely an overestimate for 2024” because of “continuing improvements in solar PV and wind turbine performance”. For solar PV, where the steel is in the supporting structures, this may be the case. However, for wind turbines, in my article I cite several recent papers that show that the steel intensity is actually increasing rather than decreasing, because wind turbines are getting larger and larger wind turbines need more steel per MW of installed power. Offshore wind turbines are also moving into deeper waters to the same effect. In other words, a higher performance comes at the expense of a higher steel use.
I don’t know where Statista got the data for the steel intensity of wind turbines (you do not provide a link) but they state that off-shore wind turbines have lower steel intensity than onshore turbines. This contradicts all the studies I cite about the steel intensity of wind turbines. Offshore wind turbines require much more steel for their foundations than onshore wind turbines.
You cite a report from the ETC called “Material and resource requirements for the energy transition”, which you say arrives at a lower need for steel. To judge the outcome of this document, I need to know on which data they built their conclusions. Most importantly, how much steel is required to build wind turbines? I could not find these data in the document. Furthermore, the authors clearly ignore the fact that larger wind turbines need more steel per MW (p.40/41). They also assume very optimistic data for the recycling of steel (p.36&49), completely ignoring the problem of the steel-energy nexus that my article raises. That greatly lowers the need for steel in their scenarios. Finally, this document is not a scientific paper. It is a report made by a think tank mostly consisting of businesses and finance institutions involved in the energy transition.
You criticize the study of Vidal et al, because it is over 10 years old, and argue that there has been much evolution in wind turbine and solar PV since then. However, again: the evolution has not lowered the need for steel, on the contrary: wind turbines are getting larger and therefore require more steel per installed MW. Vidal’s steel intensity for wind turbines (130 ton steel per megawatt of installed power) is in fact obsolete as newer wind turbines are larger and have a higher steel intensity (see the section “Steel and wind power” in my article).
You then cite a more recent paper of which Vidal is one of the co-authors, and which argues that recycled steel from decommissioned fossil fuels infrastructures could meet the cumulative demand of future low-carbon technologies. However, for this to happen low carbon power sources need to replace fossil fuel power plants. In reality, wind turbines and solar panels are not replacing fossil fuel power plants, they are added to the existing energy mix to meet the growing energy demand. The study you cite assumes that energy demand, rather than further increasing, will actually fall by 7% between 2020 and 2050. That means it underlines the conclusions of my “low-tech solution.” In the discussion, the authors state that “We observe that the increase of fossil fuel demand in the [other scenarios] hinders any secondary steel use in the construction of low-carbon infrastructures”. See also their section 5.3, where they list several other obstacles to recycling the steel used in the fossil fuel infrastructure, even in a degrowth scenario.
You argue that although hydrogen-based production consumes 10 times more energy than scrap-based production, replacing blast furnaces with hydrogen-based production would still amount to a halving of energy use. That is correct. But is a halving of energy use enough? By the time this hydrogen infrastructure is in place, the steel output would have doubled again and this efficiency advantage will be erased. Also, it will take a lot of time to convert the global steel industry to hydrogen, while my low-tech solution could be applied immediately.
All this is not to say that my numbers should be taken as the absolute truth. It’s one thing to describe the problem of the steel-energy nexus (about which we agree), it’s quite another to put precise numbers on it. Considering the many factors involved, it can only be an estimation based on the best available data at the moment.
M. E. Selous
Hi Kris,
Thanks for taking the time to respond to my comment. I appreciate the exchange. We could keep discussing many of the points you raised in objection. However as you pointed out I made a glaring error in my calculations for offshore wind, and did not provide sources for you to scrutinize. I therefore feel the need to correct this.
Statista’s data was collected from a series of IRENA reports: For solar: Renewable Energy Benefits: Leveraging Local Capacity for Solar PV, June 2017, page 13 https://www.irena.org/-/media/Files/IRENA/Agency/Publication/2017/Jun/IRENA_Leveraging_for_Solar_PV_2017.pd Data collected by IRENA itself For onshore wind: Renewable Energy Benefits: Leveraging Local Capacity for Onshore Wind, June 2017, page 21 https://www.irena.org/-/media/Files/IRENA/Agency/Publication/2017/Jun/IRENA_Leveraging_for_Onshore_Wind_2017.pdf Data taken from Vestas’ 2015 annual report For offshore wind: Renewable Energy Benefits: Leveraging local capacity for offshore wind, May 2018, page 23 https://www.irena.org/-/media/Files/IRENA/Agency/Publication/2018/May/IRENA_Leveraging_for_Offshore_Wind_2018.pdf Data compiled from the following sources, going back to 2011 at most: Birkeland, C. (2011), Assessing the Life Cycle Environmental Impacts of Offshore Wind Power Generation and Power transmission in the North Sea, Norwegian University of Science and Technology, daim.idi.ntnu.no/ masteroppgaver/006/6222/masteroppgave.pdf Siemens (n.d.), “A clean energy solution–from cradle to grave: Offshore wind power plant employing SWT-6.0-154”, www. siemens.com/content/dam/internet/siemens-com/global/ market-specific-solutions/wind/brochures/epd-swt-6-0-154.pdf (accessed 29 March 2018) The Crown Estate (n.d.), “Offshore processes in the development, installation and operation of a Round 3 wind farm”, www.thecrownestate.co.uk/media/5516/round_3_ windfarm_offshore_processes.pdf (accessed 29 March 2018) Dillinger Hütte GTS (n.d.), “Solutions in steel for offshore wind energy installations”, Alpha Ventus, The World’s First High Seas Windfarm, www.dillinger.de/imperia/md/ content/dh/referenzen/windkraft/offshorewind_englisch.pdf (accessed 29 March 2018) Kaiser, M. J. and B. F. Snyder (2012), Offshore Wind Energy Cost Modeling: Installation and Decommissioning, SpringerVerlag, London
Unfortunately, I incorrectly read the data which I pulled from Statista. The correct figures are in fact 56 tons of steel for 1MW solar, 117 tons of steel for 1MW onshore wind, and 550 tons of steel for 1MW offshore wind.
Let’s take once again the IEA’s net-zero scenario: 17,600 GW of solar and 6,700 GW of wind capacity addition. The IEA’s 2023 world energy outlook is a little fuzzy on how much wind capacity addition will come from offshore. From the projected shift in the energy mix from today to 2050 it looks to be about 40%. Assumptions are similar in the ETC report I cited.
This gives us the following rough estimate for cumulative steel demand till 2050: 56 * 17,600 * 10^3 + 117 * 6,700 * 0.60 * 10^3 + 550 * 6,700 * 0.40 * 10^3 = 2,930 Mt steel
All in all the estimate has not grown so much in relative terms, although my numbers for offshore wind were indeed wildly incorrect and I am surprised that I did not notice this first.
Much of the data I pulled from Statista seems to be from around the same period as O. Vidal et al.’s 2013 paper. My comments on the paper’s obsoleteness are therefore misplaced. However, I will point out that I felt the need to do this calculation in the first place because that 2013 paper is a short commentary piece, mostly focused on policy, and has no explanation for the figures it proposes.
Kind regards, Marrec
Mario Stoltz
Dear Kris,
I believe this is your single most important article to date in all of the years I am a reader of your website(s). Thank you so much for making this available. I believed until this morning that I was fairly aware of the challenges related to climate change, but this one indeed makes everything so much more concrete and shows the correlations. A true eye opener, though also with a fairly bleak outlook. I fear the political incentive for putting in place the necessary regulation is low.
So, let us all go lobbying in our own local environments – I certainly will.
Doug Wilson
Hello Kris,
This was a fascinating article and I appreciate the analysis. I share M. E. Selous’ concern that this overstates the issue with steel production in the long run. I had to convert the 20 GJ/t current production figure to kWh to make the comparison but came to the same observation that hydrogen-based production was around half the energy intensity of blast furnace production.
“But is a halving of energy use enough? By the time this hydrogen infrastructure is in place, the steel output would have doubled again and this efficiency advantage will be erased. Also, it will take a lot of time to convert the global steel industry to hydrogen, while my low-tech solution could be applied immediately.”
To say the efficiency advantage would be “erased” seems to make an equivalence between burning coal and burning green hydrogen because they both produce energy. It should be excellent news that switching to a carbon-free production method will not demand more energy than what is used now. Obviously, the transition would be difficult, so I see the concern on steel intensity.
The first thing that came to mind when reducing steel usage is framing for housing and light commercial construction. In the United States, most of this is timber-framed, while I understand these are frequently made out of steel beams or concrete in many other places. While poor American construction quality is sometimes lampooned by Europeans, a timber-framed building built with proper acoustic isolation and something a little better than the bottom-of-the-barrel American code standards is plenty comfortable and durable. I’m curious which other specific and obvious opportunities for reducing steel consumption you see.
Rob de Waard
Hi
Very good article. Questionnaires. How much iron could be replaced by wood? Note. In the building industry wood would give sígnificant co2 reduction because of necessary forestry and cement reduction.
Great magazine.
Ys Rob de Waard